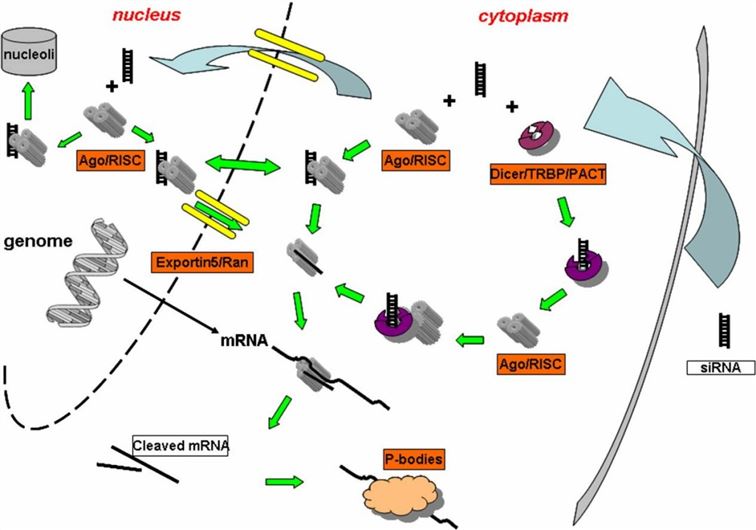
In 2000, Science magazine recognized “Revisiting RNA” as one of the top ten breakthroughs in technology, highlighting siRNA, shRNA, and miRNA as stars in the RNA field. These RNAs are pivotal in RNA interference (RNAi), a phenomenon where specific short double-stranded RNAs induce gene silencing. Andrew Z. Fire and colleagues demonstrated that double-stranded RNA’s inhibitory effect surpasses that of antisense RNA, coining the term RNAi.
RNAi garnered significant scientific acclaim, named a top ten breakthrough in 2001 and 2002 by “Science” and “Nature,” respectively. In 2003, small RNA research continued its prominence, once again making the “Science” top ten list. Andrew Z. Fire and Craig C. Mello were awarded the Nobel Prize in Physiology or Medicine in 2006 for their research on RNAi mechanisms.
In 2018, gene silencing drugs based on RNAi principles successfully entered the market, reaffirming their status as a top-ten breakthrough. This underscores the profound scientific significance of RNAi research, where siRNA, shRNA, and miRNA play crucial roles.
siRNA
siRNA, short for small interfering RNA, refers to a class of double-stranded RNA molecules approximately 21-23 nucleotides in length (literature reports suggest a range from 19 to 25 nucleotides). These molecules typically possess two unpaired nucleotides at the 3′ end and function through the RNA interference mechanism to induce gene silencing of target genes.
Figure 1. Structure of siRNA
shRNA
Short hairpin RNA (shRNA) is a type of short double-stranded RNA structure with a specific stem-loop sequence, typically ranging in length from 19 to 25 nucleotides. This structure can be introduced into cells via vectors and undergo enzymatic cleavage to form siRNA within the cell. Subsequently, these siRNAs achieve precise modulation of target genes through the RNA interference mechanism. This technology provides a robust tool in modern molecular biology research, aiding in the deeper understanding of the intricate mechanisms governing gene expression regulation.
Figure 2. Structure of shRNA
miRNA
MicroRNAs (miRNAs) are small RNA molecules ubiquitous in eukaryotes, typically measuring approximately 22 nucleotides in length (ranging from 21 to 24 nucleotides). Mature miRNAs exist as single-stranded RNA molecules and primarily regulate the gene expression of target genes through mRNA degradation or inhibition of protein translation. They are widely distributed across eukaryotic organisms and play crucial roles in various biological processes.
Figure 3. Structure of miRNA
From a structural and definitional perspective, an in-depth examination reveals three primary similarities and distinctions among siRNA, shRNA, and miRNA:
Initially, these molecules share a characteristic in their mature form, typically spanning 20 to 25 nucleotides (nt). Specifically, siRNA manifests as double-stranded RNA (dsRNA), featuring two unpaired nucleotides at the 3′ terminus and a phosphate group at the 5′ terminus. shRNA, post-processing, transforms into siRNA, whereas miRNA ultimately adopts a single-stranded RNA structure.
Secondly, concerning their origins and structural attributes, siRNA predominantly comprises artificially synthesized linear dsRNA, whereas both shRNA and miRNA form local dsRNA structures through hairpin formation. Notably, siRNA and shRNA exhibit fully complementary double-stranded regions, while miRNA often displays imperfect complementarity.
Lastly, although shRNA and miRNA exhibit some structural resemblances, functionally, they align more closely with siRNA. Specifically, post-Dicer enzyme cleavage within cells, shRNA can generate siRNA and exert interference via the siRNA pathway. In contrast, miRNA regulates target genes through a distinct pathway.
Despite their shared traits and potential for substitutability under specific contexts, each plays a distinct role in RNA interference mechanisms. Subsequent sections will delve into a detailed analysis of their specific associations and divergences.
Differences and Connections among siRNA, shRNA, and miRNA
shRNA, constructed via vectors to achieve siRNA in vivo, shares structural similarities with siRNA but demonstrates functional distinctions. Meanwhile, miRNA, another type of small RNA, exhibits close associations with siRNA and RNAi, yet distinct differences from siRNA are evident across multiple dimensions. Subsequent sections will delve into detailed discussions on the relationships and disparities between shRNA and siRNA, as well as miRNA and siRNA.
shRNA and siRNA
Figure 4. Schematic diagram of gene regulation by shRNA and siRNA
The figure above illustrates the fundamental mechanisms by which shRNA and siRNA regulate gene expression within cells. Despite their different pathways, they converge on a common objective. siRNA can be synthesized exogenously or derived from longer double-stranded RNA precursors. These dsRNAs enter cells through transfection and undergo a series of enzymatic processes in the cytoplasm to mature into functional siRNAs. Mature siRNAs subsequently associate with the RNA-induced silencing complex (RISC), leading to mRNA degradation through complementary base pairing.
On the other hand, shRNA relies on vectors, viruses, or Agrobacterium as carriers to enter cells via transfection or infection. Within the nucleus, shRNA transcripts form hairpin double-stranded structures and undergo processing by enzymes like Drosha to generate mature shRNAs. Afterward, mature shRNAs are transported to the cytoplasm where they are processed into siRNA by ribonucleases, entering the RNAi pathway.
In summary, while shRNA and siRNA employ distinct mechanisms for gene regulation within cells, both ultimately achieve mRNA targeting, demonstrating the diversity and flexibility of gene regulation mechanisms. Despite their functional similarities in final outcomes, shRNA and siRNA are not interchangeable.
This distinction primarily stems from several key aspects:
Transfection Pathways Variability
Initially, direct transfection of siRNA or long double-stranded RNA in animal cells shows suboptimal efficiency. Similarly, direct transfection is unsuitable for plant cells due to challenges in protoplast regeneration. In contrast, viral vectors such as lentiviruses or adenoviruses carrying shRNA exhibit markedly superior infection efficiency. Moreover, when shRNA vectors are transformed into Agrobacterium, they readily infect plant callus tissues.
Divergent Interference Mechanisms
Figure 5. siRNA interference pathway
According to the findings of Jarve et al., upon transfection into cells, long dsRNA rapidly translocates into the cell nucleus within 15 minutes. Subsequently, over the next four hours, it extensively distributes in both intact and dissociated forms throughout the cytoplasm. Within the cytoplasm, dsRNA forms complexes with Dicer, TRBP (known variably as R2D2 in Drosophila), and PACT, the latter being an activator protein. Dicer, leveraging its double-stranded RNA-specific ribonuclease III activity, efficiently processes the dsRNA into siRNA molecules typically ranging from 21 to 23 nucleotides in length, bearing two unpaired nucleotides.
These siRNA fragments subsequently associate with the RNA-induced silencing complex (RISC). Importantly, siRNAs that are meticulously designed and processed prior to cell transfection can bypass the processing by Dicer, TRBP, and PACT, directly binding to RISC. RISC primarily comprises proteins such as Argonaute-2, Dicer, and TRBP.
Following association with RISC, the two strands of siRNA separate, with one strand dissociating from the complex. The RISC complex carrying the antisense strand is responsible for identifying and guiding the complex to target mRNA, ultimately leading to the cleavage and degradation of the mRNA.
Figure 6. shRNA interference pathway
Compared to siRNA, the synthesis of shRNA occurs within the cell nucleus. Upon entering the cell via transient or stable transfection methods, the shRNA vector is initially transported to the nucleus. Inside the nucleus, shRNA is processed by the Drosha/DGCR8 complex, converting it into pre-shRNA. Subsequently, the Exportin-5 protein transports pre-shRNA into the cytoplasm. Within the cytoplasm, the Dicer complex removes the hairpin structure of pre-shRNA, thereby generating siRNA. Following this, siRNA binds to the RNA-induced silencing complex (RISC) and releases one RNA strand, enabling the complex to identify and degrade target mRNA.
Thus, it is evident that the processing pathway of shRNA post-nuclear export is broadly similar to that of dsRNA, ultimately forming a siRNA structure. However, shRNA exhibits superior characteristics in terms of stability and sustainability.
Duration of Action
Due to its transient conversion nature, siRNA cannot achieve prolonged maintenance and detection, nor does it possess the ability to be inherited by subsequent generations. In contrast, shRNA can integrate into the cellular genome, ensuring its intended effects persist in descendant cells.
Expression Levels
Within cells, siRNA levels are directly influenced by RNA quantity and transfection efficiency and decrease over time. However, shRNA can precisely control expression levels through inducible promoters and maintain sustained expression.
Off-Target Probability
Despite the high specificity of RNAi as a gene-silencing mechanism, it still exhibits specific and non-specific off-target effects. Specific off-target effects may arise from partial complementarity between the sense or antisense siRNA strands and non-target mRNA sequences, posing potential issues in both siRNA and shRNA applications. According to the literature, off-target inhibition can occur with as few as seven nucleotides of complementarity, influenced by factors such as sequence composition surrounding the complementary region, the specific location of the sequence on mRNA, and the number of copies of the corresponding sequence in mRNA.
Although chemical modifications of siRNA, such as methylation of the 2′-ribose of the second base, have been reported to effectively reduce off-target effects, caution is warranted in applying such modifications as they may decrease overall inhibitory capacity, thereby affecting the practical application of RNAi technology.
It is noteworthy that evidence suggests shRNA exhibits superior off-target effects compared to siRNA. Although the specific mechanisms behind this phenomenon require further exploration, one plausible explanation is the higher stability of shRNA transcribed in the cell nucleus, whereas siRNA may undergo degradation in the cytoplasm, leading to off-target effects.
miRNA and siRNA
According to early definitions of RNAi, miRNA was not initially included in its scope. Despite being discovered in 1993, the detailed functional studies of miRNA lagged behind those of siRNA, with RNAi definitions primarily focusing on siRNA. However, with advancements in scientific research, three consecutive articles published in Science in 2001 elucidated the characteristics and functional roles of miRNA in Caenorhabditis elegans, revealing its crucial regulatory roles in biological growth and development.
As research progressed, scientists discovered numerous similarities between miRNA and siRNA, with both sometimes utilizing the same gene-silencing pathways. Therefore, while there is no definitive literature concluding whether miRNA-mediated gene regulation falls under RNAi, many studies consider miRNA as another implementation pathway of RNAi. This article supports this viewpoint, namely that siRNA, shRNA, and miRNA each represent distinct implementations of RNAi, complementing each other to form a comprehensive framework of RNA interference.
Figure 7. RNAi pathways of miRNA and siRNA
The figure above provides a detailed overview of the specific pathways through which miRNA and siRNA execute RNAi within cells. Previously, we extensively discussed the pathway of siRNA. By comparison, it is evident that the miRNA pathway exhibits significant similarities to the shRNA pathway. Specifically, following transcription within the cell nucleus, the miRNA vector undergoes processing by the enzyme Drosha to form pre-miRNA. Subsequently, pre-miRNA is exported from the nucleus to the cytoplasm through the action of proteins such as Exportin-5. Within the cytoplasm, pre-miRNA undergoes further processing by enzymes like Dicer, which removes its hairpin structure. Afterwards, mature miRNA is loaded onto the RNA-induced silencing complex (RISC), simultaneously dissociating the other RNA strand. Subsequently, miRNA-RISC pairs with target mRNA in a manner that is not fully complementary. Ultimately, this process may lead to mRNA degradation or inhibition of its translation, thereby interfering with the expression of target genes.
It is crucial to clarify here that the prevailing view suggests that when miRNA achieves complete complementarity with mRNA, it triggers mRNA degradation. In cases of partial complementarity, miRNA binds to mRNA, thereby inhibiting protein translation. Indeed, when complementarity reaches a high level, it results in mRNA cleavage and degradation within the RNAi pathway, a process executed by Ago proteins possessing specific capabilities to cleave double-stranded RNA. However, specific literature defining the threshold for “high complementarity,” such as 99% or 89%, remains absent.
In animal cell systems, the relationship between miRNA and mRNA is predominantly partial, typically anchoring within the mRNA’s 3’UTR region. In contrast, in plant cells, complete complementarity between miRNA and mRNA is more common, often leading to mRNA degradation. Importantly, miRNA’s complementarity involves interactions not only with mRNA but also within its own stem-loop double-stranded structure. In most cases, these two complementarity relationships do not simultaneously reach a complete state; if they do, miRNA’s properties will approximate those of shRNA.